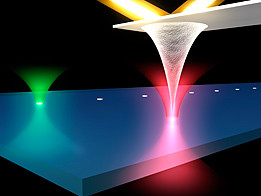
Proteins contain chemical groups that ionize (usually by either losing or gaining protons to become negatively or positively charged, respectively) at different solution acidities. In highly acidic solutions (where the pH is low), proteins tend to have positive charges, whereas in lowly acidic solutions (where pH is high), proteins tend to be negatively charged. In each case, similarly charged proteins repel each other. However, when a protein is charge neutral (that is, at its pI), it experiences the smallest repulsion from another protein, thereby causing precipitation. An everyday example of such precipitation is curdled milk. When milk pH drops to become more acidic (either through the addition of acid or the fermentation process), the protein molecules in milk, known as casein, attract one another, forming clumps or curdles.
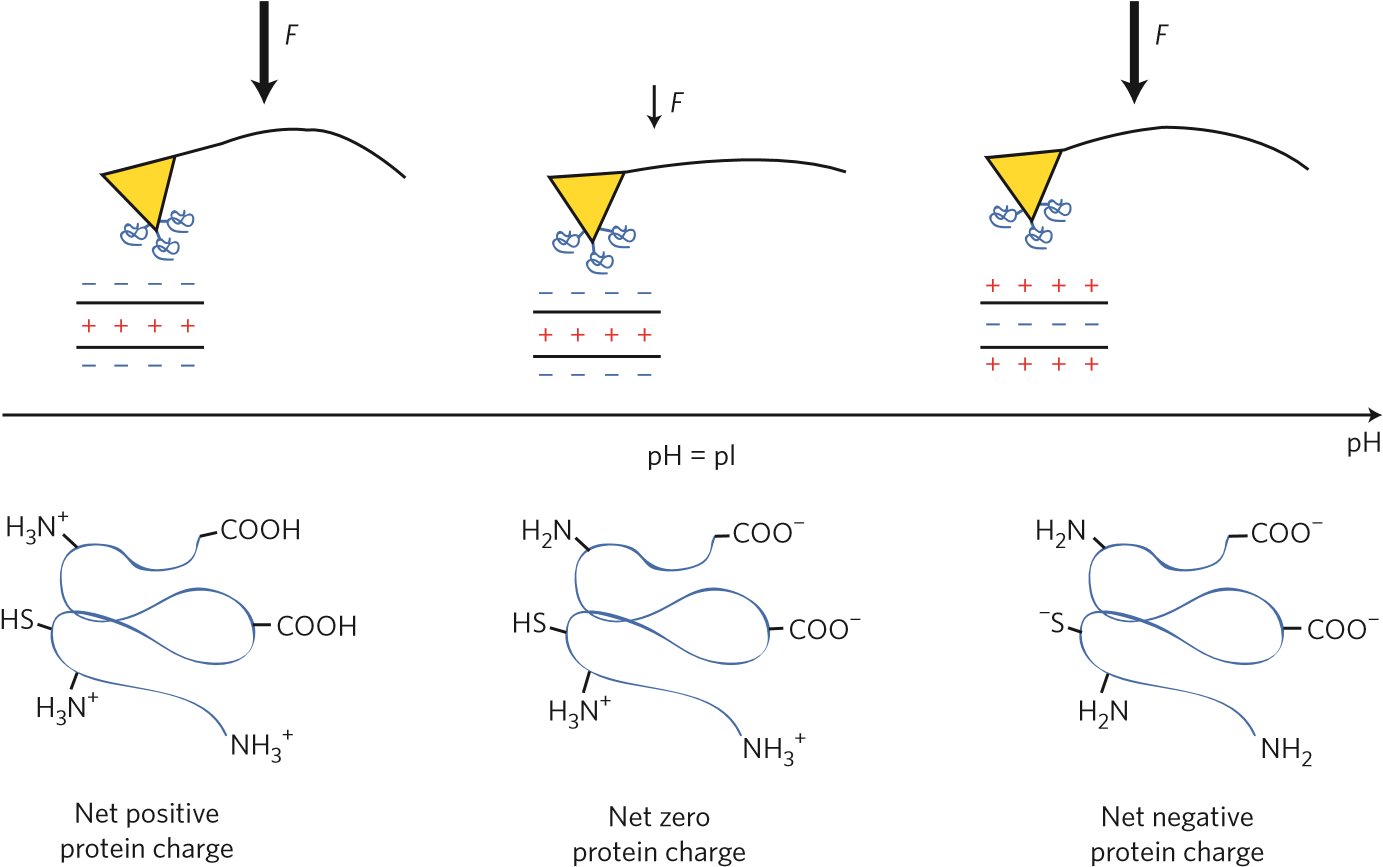
Figure 1 | Measuring the isoelectric point of a protein by atomic force microscopy. Protein (blue spirals) are attached to the atomic force microscope tip (yellow triangles). The functionalized tip interacts with the polyelectrolyte layer on a surface with a known charge. The adhesion force, F, is measured by pulling the tip away, and observing the cantilever bend. Larger arrow represents stronger force. The force dependence on solution pH reveals the protein isoelectric point, pI. At low pH, the proteins are positively charged, and are strongly attracted to a negatively charged surface. At the pI, the protein has net zero charge and experiences weaker attraction to the surface. At high pH, the protein is negatively charged and is strongly attracted to a positively charged surface.
Isoelectric points of four reference proteins — bovine serum albumin, fibrinogen, myoglobin and RNAase A — measured using the method had values comparable to those reported in the literature. Furthermore, Vancso and co-workers showed that the method could estimate the pI of a novel adhesion protein obtained from the ‘footprint’ secreted by the barnacle cyprid larvae of Amphibalanus amphitrite. Using both positively and negatively charged surfaces, the pI of this footprint protein was determined to be between 9.6 and 9.7. This protein, which forms the temporary attachment protein of the barnacle and comes in tiny quantities, is relevant in marine adhesion and fouling on ship hulls. Because the pH of seawater is about 7.8 to 8.3, this protein is expected to have a net positive charge in seawater.
Vancso and co-workers’ method has many advantages. The authors report that fewer than 1,000 protein molecules are needed to determine the pI. This means that we can now also study adhesives of other microorganisms on both natural and synthetic surfaces that come in small quantities. Furthermore, the method could potentially be used in proteomics to determine the pI of target proteins that are obtained from the digests of single cells and microdissected tissues.
Compared with gel-based isoelectric focusing and capillary isoelectric focusing methods, Vancso and co-workers’ method based on atomic force microscopy has several benefits. For example, in gel-based isoelectric focusing, it is difficult to study hydrophobic proteins because they have low solubility and do not migrate well in the gel. With the atomic force microscopy approach, proteins do not need to be highly soluble because the method uses the force of a pulling cantilever to overcome both the hydrophobic and charge interactions of the protein with the surface onto which it is adsorbed. In the case of capillary isoelectric focusing there is also a problem of protein precipitation, which may be overcome by the atomic force microscope’s ability to mechanically force the solubilization of the protein by pulling it away from the surface and into the solution with the tip. Compared with another method, where the pI is obtained by measuring the zeta potential of protein-grafted nanoparticles, the technique of Vancso and co-workers should require less sample and is suitable for insoluble proteins.
In future studies, the atomic force microscopy-based method can be further developed and used to study how added ions and macromolecules affect the attraction of proteins to hydrophobic surfaces. Furthermore, variations of this technique may allow us to study many other interfaces where a macromolecule’s pI is a key ingredient to understanding surface adhesion or protein–protein interactions. For example, to study protein adhesion on substrates with varying surface charge or acidity, which can affect the adsorption of ionizable macromolecules, one might imagine running the experiments in reverse. Instead of measuring the adhesion of a protein to a surface of known charge, one could measure the pH dependence of the adhesion of a protein with a known pI to a surface with an unknown, heterogeneous charge distribution. This approach would be valuable in quantifying protein partitioning across a surface. Done at the nanoscale, this approach could, for example, help us to understand the formation of protein coronas on surfaces exposed to serum. Such a technique might help us to uncover the origins of nanoparticle fate in living systems, which is a major current challenge to developing effective theranostic nanoparticles.